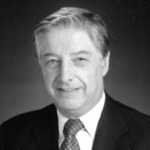
Chapter 02: Cobalt-60 and the Evolution of Radiation Therapy at MD Anderson
Files
Description
Dr. Almond explains how the use of cobalt-60 could overcome several of the issues presented by x-rays in this chapter. He then discusses the progression of radiation machinery that used cobalt-60. From Betatrons to linear accelerators, Dr. Almond describes the evolution, advantages, and disadvantages of the different early radiation machinery used to treat tumors.
Identifier
AlmondP_01_20040404_C02
Publication Date
4-4-2004
Publisher
The Making Cancer History® Voices Oral History Collection, The University of Texas MD Anderson Cancer Center
City
Houston, Texas
Interview Session
Topics Covered
The University of Texas MD Anderson Cancer Center - Devices, Drugs, Procedures; Overview; Overview; Definitions, Explanations, Translations; MD Anderson History; MD Anderson Snapshot; Understanding Cancer, the History of Science, Cancer Research; The History of Health Care, Patient Care; Technology and R&D; Patients; Patients, Treatment, Survivors
Creative Commons License
This work is licensed under a Creative Commons Attribution-Noncommercial-No Derivative Works 3.0 License.
Disciplines
History of Science, Technology, and Medicine | Oncology | Oral History
Transcript
Peter Almond, Ph.D.
But cobalt 60 seemed to be promising, because it had a high-energy gamma ray coming out of it. The average energy was over a million volts, so that’s a big advantage. The other thing that happens when you go to the higher energies, when you deposit energy with a radiation beam in the patient, the X-rays interact, set in motion the electrons, and it’s the electrons that deposit the energy. Now, if your X-rays are low energy, the electrons coming off are fairly low energy and they don’t go any distance. If you’re up in higher energy, you set electrons in motion with quite a lot of energy, which go in a forward direction and deposit their energy downstream. By the time you get to cobalt 60 energies, it takes about half a centimeter or so for the radiation dose to meet its maximum in the body. So the skin dose now is maybe 50 percent of what the maximum dose is, which is a half a centimeter. Then the dose, let’s say, ten centimeters could be 50 percent, 60 [percent].
So you do two things. You put the radiation dose deeper into the body, and at the same time, you spare the skin. Radiation reactions with cobalt 60 are much less than with the old x-ray machines. Old x-ray machines in that range, a hundred to two hundred KEV were called ortho- voltage, and you’ll see that term. It’s ortho-voltage therapy and ortho-voltage machines. We had a whole suite here of ortho-voltage machines when I first came. As I say, it was the skin dose that limited the treatment. Cobalt 60 immediately sort of said, “Well, skin dose is going to be far less,” which it was clinically. It was still there, but it was far less, and you’re putting the radiation much deeper.
The second thing is with the cobalt 60, you could get enough of it in terms of its activity to put in the machine so the output was high enough and so that you could do this. Radium just didn’t put out. You know, you didn’t get enough radium to really have the treatments over a short-enough
time. Cobalt 60, you could get enough cobalt into the unit. Treatment times were a reasonable length. Because you had enough radiation, you could move the patient about eighty centimeters to a hundred centimeters from the source, so you had a nice little distance between the source and the patients. There are various reasons you want to do that, but about a meter is about the optimum from the source, and you could get to that with cobalt 60. So cobalt 60 looked like having a lot of advantages, and Grimmett and others in the development of the cobalt 60 immediately allowed you to do a lot of things you couldn’t do.
If that was true, if you went from, say, 250,000 electron volts to one million electron volts, it could up now to ten, twenty million electron volts, really start, because now the skin dose really gets very, very low. The maximum dose is about several centimeters into the body. By the time the 50 percent dose is sixteen centimeters into the body, you can really start to put a lot of radiation dose into deep-seeded tumors, and you’re not limited by any skin reaction whatsoever. Difficulty is getting the twenty million electrons for your x-ray machine.
One of the first sort of possibilities for that was a device called the Betatrons, in which magnetic induction is used. It’s somewhat like a transformer. You have electrons going around in a circular motion inside a tube, which has got a vacuum in it. It looks like a great big doughnut and was, in fact, called the doughnut. It was about so wide [gestures]. If you changed the magnetic field while these electrons were going around, you can induce them to a going energy, and you’re changing the field and their energies increase and the radius of their motion stays the same. If you time things just right, you can get them going around many, many times picking up energy every time they go around, and you can get out to energies of twenty million and maybe even more.
A fellow by the name of [ ] Vitereau [?] in Switzerland had this idea many years before the war. Dr. [ ] Kurston [?], University of Illinois, during the war sort of developed these. I think they had implications for sort of X-raying big pieces of equipment, clearly had some sort of military applications here. At the University of Illinois, they realized that they could generate these electrons, they could strike a target, form the X-rays, and these X-rays were very high- energy X-rays. They suddenly realized that if you can make those X-rays, there’s obviously a medical application here.
In fact, they had a graduate student on the program who had a brain tumor, and they got a local radiologist to treat him with these X-rays, so that’s how that got started. So people realized there was an application here, and right after the war a number of companies, in particular Allis Chalmers in this country and another company in Europe, decided, well, maybe this was an application to be used in treatment of cancer. So betratrons were built for that purpose. We got one here.
In fact, when Grimmett came in ’49 and ’50, he was already talking to the people about getting a Betatron, and they were already making plans for that. It didn’t come until the new hospital was ready, but it came about that time. We had an old Allis Chalmers 22MEV Betatron, and it’s great advantage was you could put more dose deeper. There was no skin reaction, so you could treat deep-seated tumors. This was why [Gilbert H.] Fletcher used it primarily for external treatment of cervix cancers. He developed a box technique, one fueled from the front, one from the back, one each from either side, and cross-fired them. The trouble with the Betatron was it was fairly low output. The treatments took longer than one would like.
James S. Olson, Ph.D.
In terms of the time it took?
Peter Almond, Ph.D.
Yes. The output was around, on a good day, perhaps sixty rads a minute. Today we’ll run machines at four hundred rads a minute. But back then, your expectations were not as high, so you could get it. It was very noisy, because it had a frequency that produced a lot of noise. It was a big, big machine. It was not particularly easy to use; limited motion with the machine, but there was some. Anyhow, clearly, 20 MEV X-rays were a great advantage. So Anderson had the cobalt and they had the 20 MEV, those two machines.
The drawbacks of the Betatron were that they were big machines, they did have low output, and if you wanted to use the electrons directly, and people were beginning to think about, well, we use electrons to produce X-rays, what’ll happen if we just use the electrons themselves? It’s very, very difficult to get the electrons out of a Betatron. Can be done, and we had one machine here called the Siemens Betatron, which did it, but it was not particularly easy. We tried to get rid of the old Allis Chalmers Betatron, but you’ve got electrons which are charged with an electrical charge going around in a magnetic field, and the magnet field makes them go around in a circular motion, and that magnetic field is there. Now, if you want to get them out going straight, it’s very difficult unless you’ve got time, somehow or other, to make the electrons think the magnetic field isn’t there and come on out. But they would never come out straight. They would always be curved, and it was just a mess. Betatron inherently has the problem, low output, you can’t get the electrons out, fairly big machine.
At the same time, you can accelerate electrons in a straight line if you get them in an appropriate electromagnetic field. What happened during the war, of course, when radar got developed, they became very interested in producing very strong sources of electromagnetic radiation, which is what radar is, I guess. So two devices were developed, the Magnetron and the Klystron.
Magnetron generally is European and came out of England, and the Klystron out of the United States, but these were two devices which produced enough power in the electromagnetic radiation to have good radar installations. People knew that if you could take that electromagnetic radiation, put it into a linear structure, which is designed with a series of cavities
along it, if you did that just right, you could introduce electrons at one end along with the electromagnetic radiation. The electrons would ride the electric and then they’d get radiation down to the other end, picking up energy as they went down these little cavities, and come out with high energies at the other end. Because it was linear, they were called linear accelerators.
So you put in electromagnetic radiation at one end, along with electrons, and you run them down these cavities, and they were circular cavities put end to end all the way down. You can either use Magnetrons or Klystrons. People started to build some linear accelerators. When you first do something, you find out all the problems with it. But certainly you can do this, and now the electrons are going straight. So you can either take the electrons out and they’ll still go straight until you bend them with a magnet, or they can hit a target and produce X-rays.
James S. Olson, Ph.D.
What’s our timing, linear accelerators now, date-wise?
Peter Almond, Ph.D.
This happened right after World War II. I mean, linear accelerators and Betatrons have about the same period of birth, if you like. Certainly, for medical practices, we’re still talking in the 1940s, 1950s, I mean, the history of it.
Now, Betatrons are relatively simple machines. There wasn’t much to them, they’re just big, and if you could build them, you could build them and get good X-rays. Linear accelerators are complicated machines. There are a lot of systems, electromagnetic radiation. You’ve got to produce the electrons. You’ve got to keep a high vacuum there. They’ve got to be kept at the right temperatures, just multiple systems in linear accelerators. To get all of these working right just together took a long time to develop, and so the early linear accelerators for medical purposes although they worked, were not too reliable or successful.
James S. Olson, Ph.D.
Dr. [Luis] Delclos told me that even when we used them here they still broke down a lot.
Peter Almond, Ph.D.
He might have thought so. [laughs] It’s very important in medical applications that you know what your up time is and what kind of percentage of running time you had. You get anything below 90 percent, and you’re in trouble, and we would keep ours running. But certainly, they would break down. You don’t want them to break down at the critical time, so high reliability. Linear accelerators are difficult to get a very high . . .Until you’ve really worked out all the . . . So it took a lot longer.
We did not even go out for . . . You know, we got the first Betatron here in ’54, the next one in
’60. It was ten years later, we started in ’69 or ’68, thinking about getting linear accelerators. So it took us ten years or more before even we thought about linear accelerators, and we wrote a request for a proposal for a linear accelerator in about ’69, and the bids came in and Raytheon [Corporation] got the bid. They worked with the French company to build this device. The French had built one or two. This was the machine that the accelerating part was in a room this long [gestures], with two stationary sections, and it went through the wall into a big rotating gantry. You may have been treated on that machine, but I think you were probably treated on a next-generation machine, ultimately.
James S. Olson, Ph.D.
What I remember now is 1970 when the Tenth Congress was here, and we really wanted to get that installed by then.
Peter Almond, Ph.D.
Yes, that’s right, we did. We put that in, and these machines could go up to, if you wanted, thirty-five million electron volts. We ran ours, again, at about twenty-million electron volts, but it enabled us to get not only high-energy X-rays out, which Fletcher wanted, but it enabled us to get electrons out. The nice thing about electrons is they do just treat the surface. But because you could change the energy of the electrons, we could treat tumors right on the surface or a centimeter deep or two-centimeters deep or three-centimeters deep, we could vary. With electrons, there’s no dose beyond where you deposit their energy for the treatment. They just stop. X-rays keep going right through the body. So with X-rays, you end up giving a total dose to the patient very low, but nevertheless much more tissue gets eradiated with X-rays.
As you know, some people wonder whether that then causes later on second tumors. That’s been looked at extensively here, and I’m not sure there’s much evidence for it. Marilyn Stovall is the person that is the expert in this institution now about second tumors due to levels of radiation, and she’s looked at it. I haven’t talked to her recently about the results.
But the electrons go to the surface tumors for chest walls, for example, after mastectomies where you may have a centimeter of tissue. You’ve got lung underneath it. Lung, of course, is extremely sensitive to radiation. You overdose it, you get necrosis and fibrosis and whatever, fibrosis of the lung. So you would like the radiation to treat the chest wall, but not go into the lung. Electrons allow you to do that. When we got the electron beam, Norah [D.] Tapley was still alive, and Fletcher. We developed the techniques for treating the chest wall with electrons and sparing the lung underneath, and electrons allowed you to do that. We did a lot of those treatments, I can tell you, and set up a whole ten weeks’ worth. It also allowed you, even the super clav and the internal mammary with the electrons, because we could just put the dose where we wanted it, we put the electrons. For things like on your hand and stuff like that, it’s ideal, because you could just go down whatever length you wanted the electrons in, and it would
spare the other side of the body, whatever part you’re talking about.
So anyhow, linear accelerators sort of came along. They were slower in developing because they’re much more complex than them, but they have a much greater potential. They have higher output. You can get a much larger radiation field with them than with the Betatrons. The Betatrons are also limited to how big the field, this dimension, and you’ve got some parts of the body you want big fields. So I would say during the seventies when there were a lot of Betatrons around and there were still some Betatron companies selling Betatrons, the linear accelerator companies started to really refine the design and reliability of the linear accelerators. In particular, Varian [?], which is an American company, built a slow-energy linear accelerator.
Accelerating structure was about a foot long, and it accelerated up to four million volts and became a replacement, really, for cobalt 60. The 4 and 6 MEV linear accelerators eventually have become so reliable, much greater sort of capabilities that cobalt 60 no longer is being used in the United States. One or two places around the world in sort of developing countries use cobalt 60 because it is cheaper.
The trouble with cobalt 60 is every five years you’ve got to change the source out, and that’s expensive. Cobalt 60 decays at a half-life of five years, up until five years. After you’ve used it for about five years, the output gets so low that treatments get too long and the replacement sources cost a lot of money. Secondly, you’ve got to have a licensed person come in and do it without creating a radiation problem. So cobalt 60 after, sort of forty years of use, finally sort of replaced linear accelerators. It’s linear accelerators are safer and might have some other things about them which make them superior to cobalt 60, it’s just [?].
But Varian, they first came out with the 4 MEV linear accelerator, which was very successful, just X-rays, didn’t use electrons, but the high output, and that became a very useful machine. They also started to produce some higher energy machines, out, too, and eventually had one that went to thirty-five, but that was really too big and didn’t have [?]. But as the linear accelerators came along, Betatrons slowly disappeared, and by the late 1980s, I suspect, all the Betatron companies were out of business. There was nothing wrong with their radiation, the machines just weren’t competitive with the linear accelerators, and so they disappeared.
But the linear accelerators do produce the same kind of radiation. They do produce electrons more efficiently, do produce X-rays, outputs. You can have almost any output you like, so I’d say we ran it about 400 rads per minutes for X-rays. You could go higher, but then there’s a little danger to have it too high, and so treatments are short. Then it allows you to do all the modern things that are done with linear accelerators now, because you can put calumniators on them that are multi-leaf in shape and fields just to the shape. They’re no longer rectangle fields. You know, they’ve got these fingers that shape the field, and so if you want any shape field, and then that shape can move during the treatment since it’s computer controlled.
So you can sort of change the shape, you can rotate these linear accelerators around the patient while the treatment’s going on while the shape of the field is changing, and at the same time, you can change the intensity of the radiation coming on, so that what you do now is then it’s called Intensity Modulated Radiotherapy, IMRT. You simply rotate the beam around while doing it.
You really sort of focus all the radiation onto the tumor, not in terms only of position, but the shape of the tumor. You can do that, and if it’s close to critical structures, you can shape the radiation so that it misses the critical structures and put the radiation right where you want it.
When you consider where we were sort of when linear accelerators and all of this started to where we are today, it really is amazing. Computers have obviously made this all possible.
Recommended Citation
Almond, Peter PhD; Brunet, Lesley W.; and Olson, James S. PhD, "Chapter 02: Cobalt-60 and the Evolution of Radiation Therapy at MD Anderson" (2004). Interview Chapters. 362.
https://openworks.mdanderson.org/mchv_interviewchapters/362
Conditions Governing Access
Open